Outstanding Research
Please click the title to see the details!
∇ Functional recovery of dexterous finger movements after spinal cord injury (Isa lab)
Tadashi Isa,
Division of Behavioral Development,
Department of Developmental Physiology, NIPS
Commands for the control of voluntary movements are supposed to originate from the primary motor cortex (M1) and transmitted to motoneurons of skeletal muscles via corticofugal fibers (Lemon and Porter 1993; Lemon 2008). Corticospinal fibers make direct connections to spinal motoneurons in primates (Bernhard and Bohm, 1954). Evolution of such direct cortico-motoneuronal connections is known to parallel the development of hand dexterity, especially independent control of individual digits (Heffner and Masterton 1975; 1983). Patients with stroke or spinal cord injury often exhibit paralysis of skeletal muscles, especially, those in distal parts of extremities. Experimental lesion of bilateral brain stem pyramid in macaque monkeys were also shown to result in permanent impairment of dexterous hand movements in monkeys (Lawrence and Kuypers 1968). Based on these observations, it is generally supposed that the direct cortico-motoneuronal connection is the basis of independent digit control. However, recently we have found that in addition to the direct cortico-motoneuronal pathways, there exist a indirect pathway from the motor cortex to hand motoneurons mediated via spinal cord interneurons (propriospinal neurons) whose cell bodies are located in the C3-C4 segments of the cervical spinal cord (Alstermark et al. 1999; Isa et al. 2006)(Fig.1).
Recently we found that transection of the direct cortico-motoneuronal pathway at the mid-cervical segment of the spinal cord in the macaque monkey results in a transient impairment of precision grip but their dexterous finger movements recover within a week to a few months (Sasaki et al. 2004)(Fig.2,3). These results suggest that even if the direct cortico-motoneuronal connections from the M1 are damaged, remaining neural pathways that survived the damage might compensate for the ability of dexterous digit movement can recover. Such mechanism is important for functional recovery through neuron-rehabilitation but it is not clear which neural pathways actually compensates for the impaired function after the brain and spinal cord injury. We use the non-human primate models to study the cortical and subcortical neuronal mechanism underlying the functional recovery after the brain and spinal cord injury.
Combination of brain imaging with positron emission tomography (PET) suggest that the activity of the bilateral primary motor cortex is increased during the early recovery stage and the activity is increased in more extensive regions of the contralesional M1 and bilateral ventral premotor cortex (PMv) during the late recovery stage (Fig. 4). Contribution of these areas to the recovery has been proved by and reversible pharmacological inactivation of motor cortical regions using microinjection of muscimol, a GABAA receptor agonist (Fig. 5). These changes in the activation pattern represent involvement of different cortical regions in different stages of functional compensation after spinal-cord injury (Nishimura et al. 2007).
Moreover, we have found that gene expression change at the cortical level during the functional recovery. We analyzed the expression of GAP-43 mRNA. GAP-43 is a protein related to neurite extension. In-situ hybridization study has shown that the expression of GAP-43 mRNA was enhanced in layers II/III of the M1, PM and the primary sensory cortex (S1) on bilateral side and large cells, presumably corticofugal neurons, in bilateral M1 (Higo et al. 2009)(Fig. 6). These results suggested the plastic change occurred on the association networks among PM, M1 and S1 and descending pathways from M1, which well matched the results of the PET study.
Furthermore, to study the change in the neural network property during the recovery process, we recorded the focal field potentials in the M1 and EMG activities of various hand/arm muscles and analyzed the coherence between the cortical activities and EMG activity (cortico-muscular coherence; CMC) and coherence between hand/arm muscle activities (musculo-muscular coherence; MMC). CMC at β–band (peak at 17Hz), which was observed in one of the monkeys disappeared and never recovered even after the functional recovery (3 months postoperatively). On the other hand, MMC at γ–band (peak at around 30 Hz), which was never observed preoperatively, became evident and prevailed widely among the hand⁄arm muscles and increased as the recovery progresses. These results suggest that subcortical common oscillator compensates for the dexterous finger movements after the lesion of the direct cortico-motoneuronal pathway (Nishimura et al. 2009)(Fig. 7).
In this way, our laboratory integrates information obtained by applying a variety of experimental techniques, such as behavioral analysis, electrophysiology, pharmacological manipulation, brain imaging, molecular biology to understand the functional recovery from partial injury of the spinal cord. Such information should be valuable for future development of effective neuro-rehabilitation for the patients suffering from the spinal cord injury.
References:
- Alstermark B, Isa T, Ohki Y, Saito Y. 1999. Disynaptic pyramidal excitation in forelimb motoneurons mediated via C3-C4 propriospinal neurons in the Macaca fuscata. J Neurophysiol 82:3580-3585.
- Bernhard CG, Bohm E. 1954. Monosynaptic corticospinal activation of fore limb motoneurones in monkeys (Macaca mulatta). Acta Physiol Scand 31: 104-112.
- Heffner R, Masterton B. 1975. Variation in form of the pyramidal tract and its relationship to digital dexteriry. Brain, Behavior and Evolution, 12: 161-200.
- Heffner RS, Masterton RB. 1983. The role of the corticospinal tract in the evolution of human digital dexterity. Brain, Behavior and Evolution, 23: 165-183.
- Higo N, Nishimura Y, Murata Y, Oishi T, Yoshino-Saito K, Takahashi M, Tsuboi F, Isa T. 2009. Increased expression of the growth-associated protein 43 gene in the sensorimotor cortex of the macaque monkey after lesioning the lateral corticospinal tract. J Comp Neurol 516: 493-506.
- Isa T, Ohki Y, Seki K, Alstermark B. 2006. Properties of propriospinal neurons in the C3-C4 segments mediating disynaptic pyramidal excitation to forelimb motoneurons in the macaque monkey. J Neurophysiol 95:3674-3685.
- Lawrence DG, Kuypers HG. 1968. The functional organization of the motor system in the monkey. I. The effect of bilateral pyramidal lesions. Brain 91: 1-14.
- Lemon RN, Porter R. 1993. Corticospinal Function & Voluntary Movement. Oxford Univ Press.
- Lemon RN. 2008. Descending pathways in motor control. Ann Rev Neurosci 31 : 195-218. Murata Y, Higo N, Oishi T, Yamashita A, Matsuda K, Hayashi M, Yamane S. 2008. Effects of motor training on the recovery of manual dexterity after primary motor cortex lesion in macaque monkeys. J. Neurophysiol. 99: 773-786.
- Nishimura Y, Onoe T, Morichika Y, Perfiliev S, Tsukada H, Isa T. 2007. Time-dependent central compensatory mechanism of finger dexterity after spinal-cord injury. Science, 318: 1150-1155.
- Nishimura Y, Morichika Y, Isa T. 2009. A common subcortical oscillatory network contributes to recovery after spinal cord injury. Brain 132: 709-721.
- Sasaki S, Isa T, Pettersson L-G, Alstermark B, Naito K, Yoshimura K, Seki K, Ohki Y. 2004. Dexterous finger movements in primate without monosynaptic corticomotoneuronal excitation. J Neurophysiol 92:3142-3147.
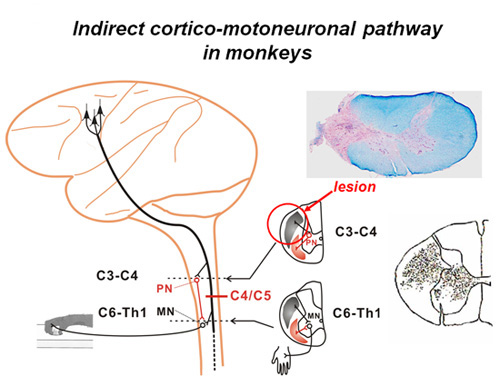
Direct and indirect cortico-motoneuronal pathway and the extent of CST lesion
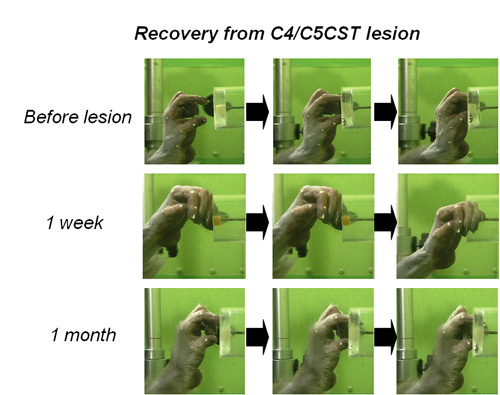
Time course of functional recovery of finger dexterity; photoimages
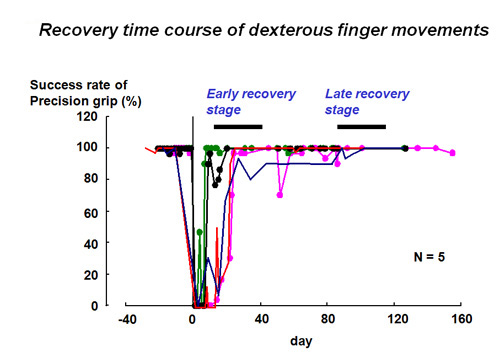
Time course of functional recovery of finger dexterity; success rate
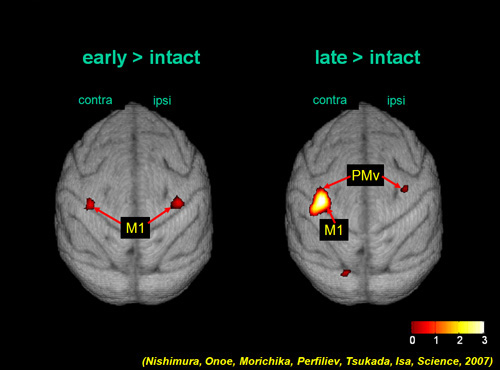
The areas whose activity increased during early (1-2 months after injury; left) and late (3-4 months after injury; right) recovery period
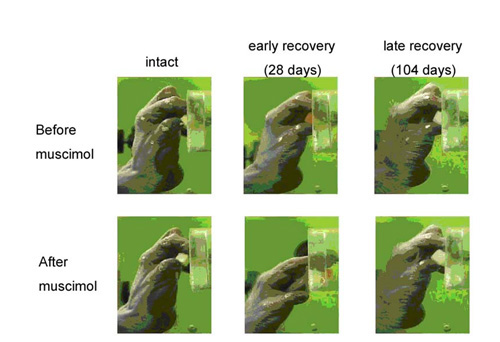
Effect of muscimol into the ipsilesional M1 hand area at the intact state, early (28 days after injury) and late (104 days) recovery period
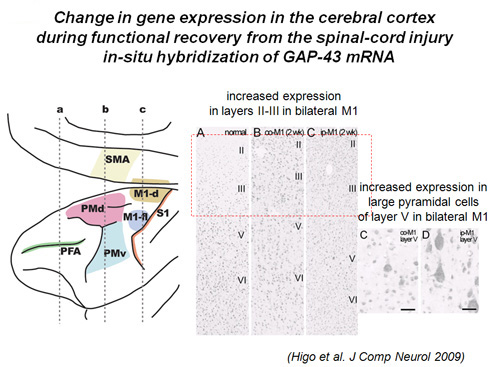
Increased expression of GAP-43 mRNA as revealed by in-situ hybridization in contralesional and ipsilesional M1
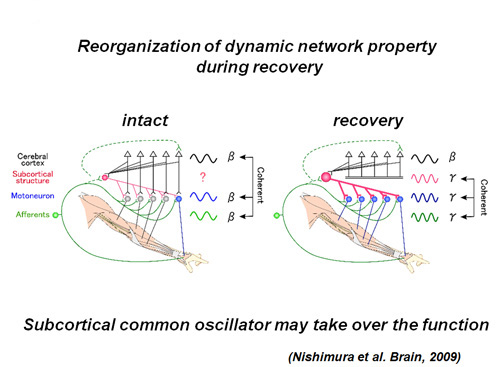
Schematic drawing of change in dynamic properties of the network operation before and after injury. Before injury, β-band cortico-muscular coherence was predominant (left). After the recovery from the lesion, γ-band musculo-muscular coherence prevailed among a variety of muscles from proximal to distal (right)
∇ Disruption of LGI1-linked synaptic complex causes abnormal synaptic transmission and epilepsy (Fukata lab)
Masaki Fukata,
Division ofMembrane Physiology,
Department of Cell Physiology, NIPS
Epilepsy, affecting 1% of the population, is a poorly understood and treated disease. So far, more than a dozen human epileptic genes have been reported, and most of them encode ion channels (channelopathy concept for epilepsy) (Ref 1). Although various mutations of a secreted LGI1 ligand were reported in an inherited form of human epilepsy, ADPEAF (autosomal dominant partial epilepsy with auditory features) (Ref 2), the in vivo function and the direct role of LGI1 in epileptogenesis remain unclear. In 2006, we identified LGI1 and its receptor ADAM22 as constituents of PSD-95-associated protein complex (Ref 3). Here, we generated LGI1 knockout mice and found that loss of LGI1 in mice causes specific lethal epilepsy (Ref 4). Taking advantage of in vivo-TAP (tandem affinity purification) method, we found that secreted LGI1 acts on two epilepsy-related receptors, ADAM22 and ADAM23, in the brain (Fig. 1). We propose that LGI1 may organize a trans-synaptic protein complex including presynaptic potassium channels (Kv1) and postsynaptic AMPA type glutamate receptor scaffolds (Fig. 2). This LGI1-mediated cell-cell interaction is unique in that the amount of secreted LGI1 may determine strength of the interaction. Such ligand-dependent cell-cell contact machinery could provide an important mechanism for regulating synaptic transmission. Our results show that LGI1 is a novel antiepileptogenic ligand that regulates brain excitation.
References:
-
Steinlein OK: Nat Rev Neurosci (2004) 5: 400-408
Kalachikov Sh, et al: Nat Genet (2002) 30: 335-341
Fukata Y, et al: Science (2006): 313: 1792-1795
Fukata Y, et al: Proc Natl Acad Sci USA (2010) 107: 3799-3804
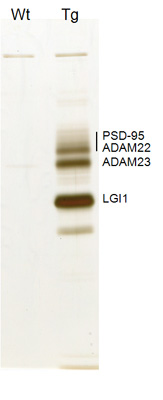
Identification of LGI1-containing protein complex in the brain. In vivo LGI1-associated protein complex purified from the transgenic mice expressing LGI1 tagged with Flag and His x6 (Tg). Wild type (Wt) mice were used as a negative control. Ref. 4
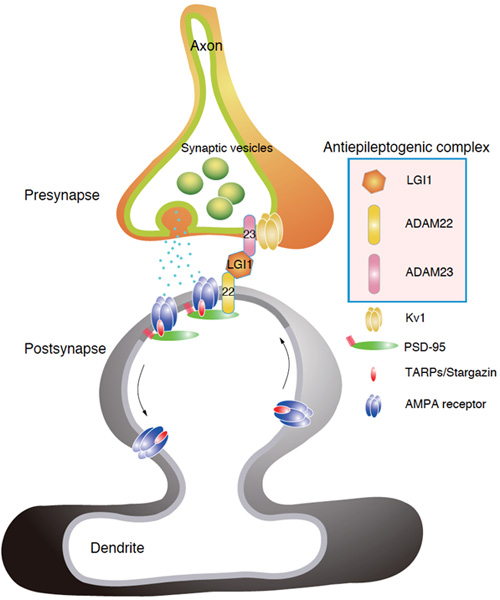
Proposed model. Loss of any one constituent of LGI1-linked synaptic protein complex causes the common epileptic phenotype in mice. LGI1 functions as an antiepileptogenic ligand that may organize a trans-synaptic protein complex and finely tune synaptic transmission.